Meiosis, a term derived from the Greek word meaning “to lessen,” is a fundamental process in eukaryotic cells that reduces the number of chromosomes. This intricate cellular division is crucial for sexual reproduction in plants, animals, and fungi. Meiosis transforms a cell with a double set of chromosomes (diploid) into cells with a single set (haploid), ultimately producing germ cells or spores. These haploid cells are essential because they unite during fertilization to create a diploid zygote, thereby maintaining the species’ characteristic chromosome number across generations. Let’s delve into the mechanisms, phases, and, most importantly, the purpose of meiosis.
The Crucial Role of Chromosomal Reduction
Imagine human reproduction without meiosis. Humans have 46 chromosomes, organized as two sets of 23 (diploid). If egg and sperm cells were produced without chromosome reduction, each would contain 46 chromosomes. Upon fertilization, the resulting zygote would possess 92 chromosomes – double the normal amount! This drastic increase would lead to severe genetic abnormalities and be unsustainable across generations. If offspring with 92 chromosomes reproduced, their progeny would inherit 184 chromosomes, and so on. This unchecked doubling would quickly become catastrophic.
Therefore, the primary purpose of meiosis is to halve the chromosome number, ensuring that when gametes fuse during sexual reproduction, the offspring inherit the correct, species-specific number of chromosomes. This chromosomal reduction is vital for the continuity and genetic stability of sexually reproducing species.
Maintaining Consistent Chromosome Number Across Generations
Before meiosis begins, the cell’s chromosomes, housed within the nucleus, undergo replication. This replication is similar to the initial step in mitosis. Meiosis, however, takes a different path, ultimately yielding four daughter cells, each with half the number of chromosomes as the original parent cell. In simpler terms, it generates four haploid cells from a single diploid cell.
To understand the chromosome count, remember that “haploid” (n) refers to one set of chromosomes, while “diploid” (2n) signifies two sets. Interestingly, to achieve the final haploid state, the parent cell first becomes tetraploid (4n) before division commences. For instance, a human cell with 46 chromosomes (2n) temporarily becomes a cell with 92 chromosomes (4n). This tetraploid cell then undergoes meiosis to produce four cells, each with 23 chromosomes (n).
The initial stages of meiosis share similarities with mitosis, particularly chromosome replication and the formation of sister chromatids (identical halves of a chromosome). However, meiosis diverges significantly with the crucial event of recombination, also known as crossing over. During recombination, homologous chromosome pairs align and exchange genetic material. This exchange ensures that each chromosome in the resulting gametes carries a unique mix of genetic information.
In summary, meiosis employs recombination to produce four haploid daughter cells that are genetically distinct from the diploid parent cell and from each other. This process is fundamental to sexual reproduction and genetic diversity.
The Two Divisions of Meiosis: A Step-by-Step Breakdown
Meiosis unfolds in two sequential divisions: Meiosis I and Meiosis II. Each division comprises distinct phases, each playing a critical role in chromosome segregation and genetic recombination. Meiosis I includes prophase I, metaphase I, anaphase I, and telophase I. Meiosis II follows with prophase II, metaphase II, anaphase II, and telophase II. To navigate these phases effectively, understanding key terminology is essential:
- Bivalent: A pair of homologous chromosomes held together by a chiasma, formed during prophase I.
- Chiasma: The point of crossing over where homologous chromosomes exchange genetic material.
- Centromere: The constricted region of a chromosome where sister chromatids are joined.
- Dyad: Composed of two sister chromatids, representing half of a tetrad or a single replicated chromosome.
- Homologous Chromosomes: A pair of chromosomes, one from each parent, carrying genes for the same traits. They are similar but not identical.
- Metaphase Plate: The equatorial plane of the cell where chromosomes align during metaphase.
- Monad: A single chromatid after the separation of sister chromatids in anaphase II.
- Nuclear Envelope: The double membrane surrounding the nucleus in eukaryotic cells.
- Nucleolus: A structure within the nucleus responsible for ribosome RNA (rRNA) production.
- Sister Chromatids: Two identical copies of a chromosome, joined at the centromere, formed during DNA replication.
- Spindle Fibers: Microtubule structures extending from the cell poles, involved in chromosome movement during cell division.
- Synapse/Synapsis: The pairing and alignment of homologous chromosomes during prophase I.
- Tetrad: Also known as a bivalent, it refers to the structure formed by two homologous chromosomes, each consisting of two sister chromatids, during prophase I.
Meiosis I: Separating Homologous Chromosomes
Meiosis I is the first division, characterized by the separation of homologous chromosome pairs. Figure 1 provides a visual overview of Meiosis I. Let’s examine each phase in detail to grasp their significance and contribution to the overall purpose of meiosis.
Figure 1: The stages of meiosis I, illustrating the separation of homologous chromosomes.
1. Prophase I: The Longest and Most Complex Phase
Prophase I is a lengthy and intricate stage, subdivided into five substages: leptonema, zygonema, pachynema, diplonema, and diakinesis (Figure 2). It is defined by three key events: chromatin condensation into visible chromosomes, synapsis of homologous chromosome pairs, and crossing over (recombination) between these synapsed chromosomes.
Figure 2: Substages of prophase I in meiosis, highlighting the intricate events of chromosome pairing and recombination.
Leptonema (Leptotene): Chromatin begins to condense, making chromosomes visible as thread-like structures. The crucial homology search initiates, where chromosomes start seeking their homologous partners.
Zygonema (Zygotene): The homology search continues, and homologous chromosomes begin to align roughly, initiating synapsis. This pairing process leads to the formation of bivalents, and the synaptonemal complex, a protein structure that facilitates synapsis and crossing over, starts to assemble.
Pachynema (Pachytene): Synapsis is completed as the synaptonemal complex fully develops between homologous pairs, resulting in tight pairing. At this stage, each bivalent is clearly composed of four chromatids, known as a tetrad. Crossing over or recombination occurs between non-sister chromatids within the tetrad, exchanging genetic material. This is a critical step for generating genetic diversity.
Diplonema (Diplotene): Sister chromatids within each homologous chromosome pair begin to separate. However, non-sister chromatids remain connected at chiasmata, the points where genetic exchange occurred during crossing over.
Diakinesis: Chromosomes continue to condense further and separate, but they remain linked at the chiasmata. The chiasmata move towards the ends of the chromatids in a process called terminalization. The nuclear envelope and nucleolus break down. Finally, centromeres attach to spindle fibers, preparing for alignment at the metaphase plate. Chromosomes remain in pairs as tetrads.
2. Metaphase I: Alignment at the Metaphase Plate
Metaphase I is similar to metaphase in mitosis. Spindle fibers, emanating from opposite poles of the cell, attach to the centromeres of each tetrad. These fibers then maneuver the tetrads to align along the metaphase plate, ensuring that each pole receives one chromosome from each homologous pair.
Figure 3: Tetrad alignment during metaphase I, ensuring proper segregation of homologous chromosomes.
3. Anaphase I: Separation of Homologous Chromosomes
In Anaphase I, a crucial distinction from mitosis emerges. Instead of sister chromatids separating, homologous chromosome pairs (dyads) within each tetrad separate. This separation, known as disjunction, sees each dyad being pulled towards opposite poles of the cell. Anaphase I concludes when each pole contains a haploid set of chromosomes, each consisting of two sister chromatids.
Figure 4: Separation of homologous chromosome dyads during anaphase I, reducing the chromosome number.
4. Telophase I: Formation of Haploid Daughter Cells
In some organisms, Telophase I marks the formation of a nuclear membrane around the dyads at each pole. This may be followed by a brief interphase period before Meiosis II commences. In other organisms, Telophase I is skipped entirely, and the cell proceeds directly to Meiosis II. Cytokinesis, the division of the cytoplasm, typically occurs during Telophase I or shortly after, resulting in two haploid daughter cells.
Image Source: Wikimedia Commons
Figure 5: Nuclear membrane formation around chromosome dyads during telophase I, resulting in two haploid cells.
Meiosis II: Separating Sister Chromatids
Meiosis II closely resembles mitosis. It is the second division, where sister chromatids within each dyad are separated, similar to what happens in mitosis. Figure 6 provides an overview of Meiosis II following Telophase I.
Figure 6: Stages of meiosis II, leading to the formation of four haploid daughter cells.
1. Prophase II: Preparing for Sister Chromatid Separation
In Prophase II, sister chromatids, now organized as dyads, remain connected at the centromere and positioned in the center of the cell. Chromosome condensation and nuclear envelope breakdown are generally not required as these events may have already occurred during Meiosis I or the transition to Meiosis II.
Image Source: Wikimedia Commons
Figure 7: Sister chromatids joined by centromeres during prophase II, ready for separation.
2. Metaphase II: Alignment of Sister Chromatids
Spindle fibers attach to the centromeres of each sister chromatid dyad. These fibers align the dyads along the metaphase plate, with each sister chromatid facing opposite poles of the cell.
Image Source: Wikimedia Commons
Figure 8: Metaphase plate formation in meiosis II, setting the stage for sister chromatid separation.
3. Anaphase II: Separation of Sister Chromatids
During Anaphase II, spindle fibers shorten, pulling sister chromatids apart at the centromere. Each separated sister chromatid (now termed a monad or chromosome) is drawn towards opposite poles of the cell.
Image Source: Wikimedia Commons
Figure 9: Sister chromatids being pulled apart during anaphase II, creating individual chromosomes.
4. Telophase II: Formation of Four Haploid Gametes
Finally, in Telophase II, chromatids (monads) reach the poles of the cell. Cytokinesis follows, where nuclear membranes form around each set of chromosomes, and the cell divides. This division results in two haploid cells from each of the cells that entered Meiosis II. The overall outcome of meiosis is the formation of four genetically distinct haploid gametes, ready for potential fusion during sexual reproduction to form a diploid zygote.
Image Source: Wikimedia Commons
Figure 10: Four haploid daughter cells formed at the end of telophase II, completing meiosis.
Key Mechanisms and Purpose of Meiosis: A Recap
The intricacies of meiosis can be demanding to follow. Here’s a summary highlighting key points from both divisions. Recombination during Prophase I involves homologous chromosomes exchanging segments of their DNA. This exchange, occurring at chiasmata, ensures that each chromosome in the resulting gametes carries a unique combination of maternal and paternal genes.
Figure 11: Illustration of genetic material exchange during meiosis and the resulting genetically diverse gametes.
Why is Meiosis Crucial in Biology? Three Key Reasons
Meiosis holds immense significance in biology for three primary reasons:
-
Enabling Sexual Reproduction in Diploid Organisms: Meiosis is indispensable for sexual reproduction in diploid organisms. It reduces the chromosome number from diploid to haploid, producing gametes that can fuse to form a diploid zygote, maintaining the species’ chromosome number across generations.
-
Generating Genetic Diversity: The crossing over or recombination of genes during Prophase I is a powerful mechanism for creating genetic diversity. It shuffles alleles between homologous chromosomes, producing novel combinations of genes in gametes. This genetic variation is essential for adaptation, evolution, and the long-term survival of species.
-
Facilitating Repair of Genetic Defects: Recombination during meiosis can also contribute to the repair of genetic defects. If a harmful allele exists on one homologous chromosome, crossing over might replace it with a healthy allele from the other homologous chromosome, potentially leading to healthier offspring.
1. Enables Sexual Reproduction of Diploid Organisms
As previously discussed, meiosis is the fundamental process that makes sexual reproduction possible in diploid organisms. By reducing the chromosome number, it creates haploid gametes that can unite to form a diploid zygote, ensuring the continuation of the species with the correct chromosome count.
2. Enables Genetic Diversity: The Engine of Evolution
The genetic recombination that occurs during meiosis is a cornerstone of genetic diversity. By mixing maternal and paternal genes, meiosis generates gametes with unique genetic makeups. This variability within a population is crucial for its resilience. Genetic diversity acts as a buffer against environmental changes, diseases, and other challenges. Without recombination and the resulting diversity, populations would be genetically uniform, making them highly vulnerable to extinction. Diversity ensures that within a population, some individuals will possess traits that allow them to survive and reproduce even when faced with adversity, securing the species’ survival across generations.
3. Aids in the Repair of Genetic Defects
The recombination process in meiosis can also play a role in repairing genetic defects. In cases where one parent carries a defective allele for a particular gene, recombination can potentially replace this allele with a healthy version from the other parent’s homologous chromosome. This mechanism can lead to offspring inheriting a healthy gene copy, mitigating the impact of genetic defects.
Meiosis vs. Mitosis: Key Differences
Mitosis, another type of cell division, produces two genetically identical diploid daughter cells from a single diploid parent cell. In contrast, meiosis generates four genetically distinct haploid daughter cells from one diploid parent cell. These haploid cells are germ cells, specialized for sexual reproduction, while mitosis typically produces somatic cells for growth, repair, and asexual reproduction.
Meiosis is exclusive to eukaryotic organisms that reproduce sexually, whereas mitosis occurs in all eukaryotic organisms, including those that reproduce asexually.
The table below summarizes the key similarities and differences between meiosis and mitosis.
Meiosis | Mitosis | |
---|---|---|
Similarities | ||
Can only occur in eukaryotes | ||
DNA replication occurs first | ||
Production of daughter cells based on parent cell’s genetic material | ||
Means of cell replication in plants, animals, and fungi | ||
Differences | ||
Starting/Ending Cell | Starts as diploid; ends as haploid | Starts as diploid; ends as diploid |
Chromosome Number | Chromosome number is reduced | Chromosome number is conserved |
Synapsis | Chromosome pairs undergo synapsis | No synapsis occurs |
Purpose | Used for sexual reproduction | Used for growth/healing/asexual reproduction |
Nuclear Divisions | 2 nuclear divisions | 1 nuclear division |
Phases | 8 phases | 5 phases |
Daughter Cell Identity | Daughter cell not identical to parent cell | Daughter cell identical to parent cell |
Number of Daughter Cells | Results in 4 daughter cells | Results in 2 daughter cells |
Cell Type Produced | Produces germ cells | Produces somatic cells |
Organism Type | Occurs only in sexual organisms | Occurs in asexual and sexual organisms |
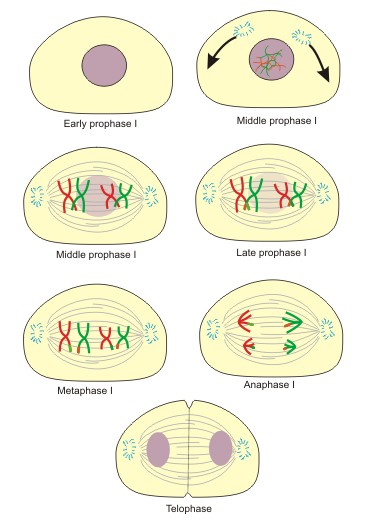
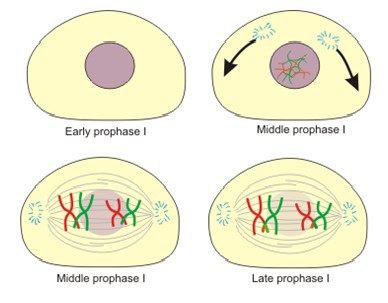
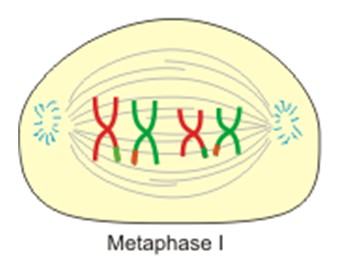
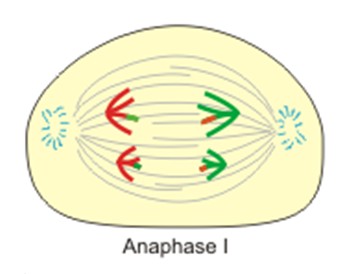
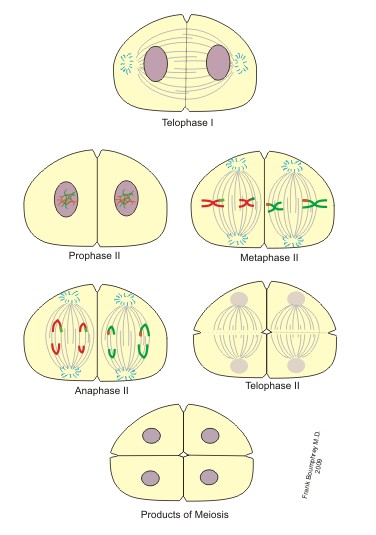
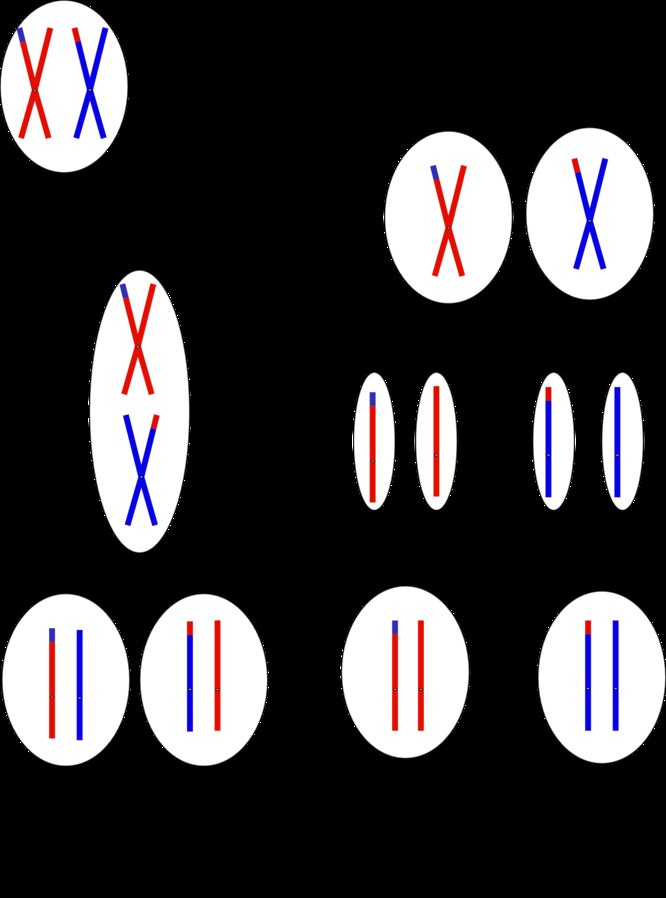
Wrapping Up: Meiosis and the Foundation of Biological Diversity
Meiosis, therefore, is the essential process of chromosome reduction, enabling the production of haploid germ cells necessary for sexual reproduction. Beyond chromosome reduction, meiosis serves the critical purpose of generating genetic diversity through recombination and potentially aiding in the repair of genetic defects.
The advantage of meiotic reproduction over mitotic reproduction lies in its ability to produce genetically diverse offspring. Mitosis generates identical copies, conserving the genetic makeup. In contrast, meiosis facilitates the expression of new traits through crossing over and recombination. This continuous generation of genetic diversity is the bedrock of adaptation and evolution. Without meiosis maintaining genetic diversity within populations, organisms would lack the capacity to adapt to changing environments, evolve, or withstand catastrophic events. A population’s genetic diversity stands as its most powerful asset in the ongoing struggle for species survival.
Explore More in Cellular and Molecular Biology
Expand your knowledge with our other articles on Cellular and Molecular Biology.
Enhance your understanding and skills with thousands of practice questions at Albert.io. Albert.io offers customizable learning experiences, allowing you to focus on areas where you need the most support. Our challenging practice questions are designed to help you achieve mastery in Cellular and Molecular Biology.
Start practicing here.
Are you an educator or administrator seeking to improve student outcomes in Cellular and Molecular Biology?
Discover more about our school licenses here.